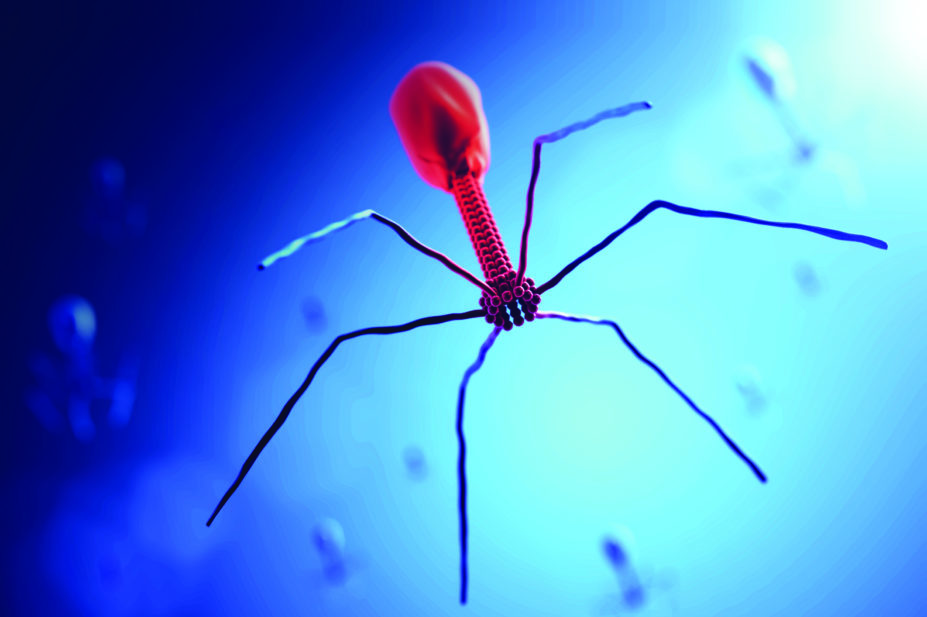
Adobe Stock
A “critical point” has been reached; we are facing a “microscopic enemy” and we risk being “cast back into the dark ages of medicine” — these are just a few of the warnings that have been made about the rise of antimicrobial resistance.
As current antibiotics slowly lose their edge, and with a lack of novel antibiotic options, exploring the potential of bacteriophages, or phages (see Figure 1) — viruses that attack bacteria — is a logical next step. Phage therapy research in the West has accelerated in recent years, and there is hope that it could provide an effective alternative to antibiotics — if difficulties around manufacturing at scale and financial incentives can be overcome.
The modus operandi of these bacteria-killers is akin to a traditional virus. After attaching to the bacteria cell through precise interactions with proteins on the cell surface, phages inject their DNA and commandeer the host cell to produce phage replicates. These replicates break open or ‘lyse’ the bacteria cell, freeing the new brood of phages and killing the bacterial host (see Figure 2).
Phages and bacteria have been engaged in this microbial war for more than three billion years, locked in a cat-and-mouse game of genetic one-upmanship, fighting for survival. The evolutionary pressure this creates explains their value as antibacterial therapeutics.
Phage particles are the majority of all biological life forms
“There’s this big number: this estimated 1031 phage particles in the world. It tells us that phage particles are the majority of all biological life forms,” says Graham Hatfull, professor of biotechnology at the University of Pittsburgh, United States, and owner of the largest collection of phages in the world. “Phages are constantly infecting bacteria and … there’s a huge number of infections going on globally, so it’s very dynamic. The whole phage population is estimated to replace itself every few days.”
Figure 1. What are bacteriophages?
Source: Shutterstock/MAG
Bacteriophages are viruses that infect bacteria. They consist of a head-like protein capsule around an RNA or DNA genome, a central shaft and leg-like appendages
This quick, relentless turnover on such an enormous scale means plenty of opportunity for mutation and genetic variety. Phage diversity offers a counter to each bacteria species, including antibiotic-resistant strains, and their capacity for fast-paced evolution means phages adapt against new resistance.
Resistance to phages is associated with reduced bacterial fitness; cleverly, the bacterial surface proteins that phages attach to often double up as virulence factors, so by removing them to evade attack by phages, bacteria downgrade their own pathogenicity[1]
. Other modifications to avoid phage attack have seen increased susceptibility of bacteria to antibiotics[2],[3]
.
Additional advantages over traditional antibiotics include phages’ efficacy against biofilms — clusters of bacteria that stick to surfaces and are protected by a matrix of sugars, proteins, and nucleic acids which are difficult for traditional antibiotics to penetrate. Phages, however, can penetrate biofilms by releasing or inducing bacteria to produce depolymerase enzymes that degrade the matrix.
Phages can also cross the blood–brain barrier, which remains a challenge for most antibiotics when targeting central nervous system infections and, unlike the more indiscriminate targeting of antibiotics, phages are highly specific to one bacteria species or even strain, governed by the compatibility of their surface protein attachment mechanism. While this removes off-target side effects common to antibiotics — which can lead to bacterial imbalances or secondary infections — it is this specificity that held them back from widespread use when first discovered more than a century ago.
Phages out, antibiotics in
Phages predate antibiotics — Sir Alexander Fleming’s discovery of penicillin was not until 1928, whereas phages were co-discovered, firstly, in 1915 by British bacteriologist Frederick Twort, and then in 1917 by Felix D’Herelle, a French-Canadian microbiologist, at the Pasteur Institute in Paris. D’Herelle, who coined the name bacteriophage, is considered the forefather of phage therapy for designing commercial phage formulations and taking them to clinical trial by 1919. Yet by the 1940s, phage therapy was out and antibiotics were in.
Figure 2. Virulent bacteriophage lifecycle
Source: Shutterstock/MAG
Bacteriophage takes over the bacterial cell, reproduces new phages, and destroys the cell
According to Elizabeth Kutter, phage researcher at the Evergreen Phage Lab in Washington, D’Herelle’s phage therapy was ahead of its time and molecular biology needed to catch up. The results of those early clinical trials were both unpredictable and unexplainable. Kutter highlights a study — the Eaton-Bayden-Jones report, published in the Journal of the American Medical Association (JAMA) in 1934 — that tarnished the scientific perspective on the therapy[4],[5],[6]
. The study reviewed over 100 reports of clinical phage use. “What they came out saying was that [those against] Staphylococcus clearly worked, but most of the others couldn’t be proven,” she says.
It is now recognised that the specificity of phage to bacteria surface proteins was behind this patchy evidence; Staphylococcus species have low surface protein variation so are successfully targeted using one type of phage, whereas species with multiple and highly varied strains, like Escherichia coli, need a more complex batch. Lacking this insight, and in the face of the JAMA critique and the success of antibiotics, phages fell out of favour and were all but forgotten.
This changed in the 1990s, after Kutter took a research trip to Moscow and discovered phage therapy flourishing unchallenged in the East; Wroclaw in Poland and Tbilisi in Georgia were strongholds of clinical phage research. But their literature was either poor compared with the demands of Western science or dismissed as a ‘Stalinistic cure’ — even, in one instance, lost in the archives of the KGB[7]
. “It’s taken until the last few years for this to be taken very seriously in this part of the world,” Kutter explains. It was not until Poland joined the European Union in 2004 that phage therapy became directly accessible to the West, and an EU-funded clinical trial, Phagoburn, investigating Pseudomonas aeruginosa infections in burn victims, was established.
We expected to see a very brilliant result of the phage group versus the control group and, instead, we observed that the phage group had improved and had a reduction in bacterial burden, but this happened slower than in the control group
Disappointingly, the results were lukewarm. “We expected to see a very brilliant result of the phage group versus the control group and, instead, we observed that the phage group had improved [and] had a reduction in bacterial burden, but [this happened] slower than in the control group,” says Patrick Jault, lead author of the Phagoburn paper published in 2018 in The Lancet Infectious Diseases
[8]
. He highlights the need to meet good manufacturing practice (GMP) regulations was not a good fit for unusual biologics like phages. For instance, storage requirements meant the concentration of the phages in formulation dropped significantly by the time they were administered, far below the intended dose.
Complex dosing
Dosing is one of phage therapy’s complexities. Although their apparent low toxicity (from prolonged exposure to them throughout human evolution) is thought to allow high doses to be used safely, phages are what is known as ‘self-dosing’; they increase their dose in situ through replication. This depends on factors such as the number of bacteria cells and length of replication cycle, which varies from 20 minutes to 24 hours depending on the phage. So, answering questions about what a minimum effective dose is, what the dose-response curve looks like, and what the best administration route or optimum dosing regimen is, is challenging. And, while generally safe, there remain unknowns around their exact immunogenic effects. These are questions easily answered for each current antibiotic, but not enough about phage pharmacokinetics is known for the same to be said for each phage.
Here, the sword that phage therapy offers against antimicrobial resistance shows its double edge; the advantage of phage diversity becomes a clinical obstacle. “You want to understand the phage you are using. It’s relevant, for example, whether they can grow and infect anaerobically,” Kutter explains. “Certainly there are places in the human body where you don’t get much oxygen, so knowing what something can do anaerobically is important.”
Financial viability
Phage-dependent properties mean phage-specific manufacturing requirements, which rule out the attractive hope of phage therapy as a quick commercial win. For instance, some are best cultured in liquid broth, others in solid agar. Some produce toxins like lipopolysaccharide, or can only be manufactured inside pathogenic hosts, which require high levels of purification.
The real problem is coming up with a convincing story that, after you spend all that time and money, you are actually going to turn a profit
But all this can be accomplished. Drew Smith, who pioneered a novel phage-based diagnostic through US Food and Drug Administration approval in 2011, says that although manufacturing comes with difficulties, “they are really just about time and money”. Phage diagnostics, of which four are currently used clinically, take advantage of phage-bacteria pairing specificity to identify an unknown bacteria strain in vitro, but the issues of cultivation, storage, and scale-up still apply. “If you have the money, it’s just work, and you can work through those challenges one by one. The real problem is coming up with a convincing story that, after you spend all that time and money, you are actually going to turn a profit.”
And this is the root of the issue: financial incentive. The commercial model for novel antimicrobials is broken, stemming from a pay-per-volume sales model. For typically short, one-off treatments with cheaper alternatives that still broadly work, a high unit price is hard to justify. Given that novel antimicrobials are often relegated to third- or fourth-line options, the return on investment that pharmaceutical companies can recoup is severely limited. Recommendations to de-link the value from the volume of units sold have been made, but there is not yet an established alternative model.
Considering this reality check, phage therapy’s best hope targets financially viable infections. Drew lists the advantages of one: Pseudomonas infections in cystic fibrosis patients. Here, “there’s one single pathogen that’s predominant. About 80% of cystic fibrosis patients go on to develop chronic Pseudomonas airway infections, and antibiotic therapy for Pseudomonas almost inevitably fails,” he explains. He adds that, in the UK, there are about 30,000–40,000 cystic fibrosis patients, which he says is “not a huge market, but it’s not that small either […] So it’s conceivable to me that that might be a clinically and economically feasible path to phage therapy.”
However, phage therapy is increasingly being backed up with research, and the scepticism that has been its hallmark is beginning to turn to excitement (see Box 1), with some researchers even pioneering next generation phages (see Box 2).
Companies working on phage therapeutics, such as France-based Pherecydes Pharma, are confident that phage therapy will eventually be an established treatment option. “The products in the pipeline [now] will be three to five years to market; in seven to ten years it will be established treatment,” estimates Guy-Charles Fanneau de La Horie, chief executive officer of Pherecydes Pharma, as clinicians become used to more targeted, precision therapies instead of blunt tools like broad-spectrum antibiotics.
But researchers warn there is still a lot of fine-tuning left to do — phages might be out of their cradle, but they are only beginning to take their first wobbly steps.
Box 1: Where are we now?
Phage therapy research in the West has accelerated in recent years. In practical terms, treatment options remain reserved for compassionate cases — but of the 29 such cases reported since 2000, half occurred after 2017[9]
. This spike follows two high-profile success stories; in 2019, Graham Hatfull, professor of biotechnology at the University of Pittsburgh, United States, had a collection of phages and the expertise that helped save the life of a girl, aged 15 years, with cystic fibrosis, whose Mycobacterium abscessus infection had persisted even after a double lung transplant. He was able to build upon the clinical knowledge of the infectious disease team at the University of California, United States, who, in 2015, had successfully tackled a highly antibiotic-resistant and deadly Acinetobacter baumannii infection by identifying a phage counteragent.
Compassionate use is spreading; France, the United States, Australia, Belgium and Poland have each established legal precedence of approval for compassionate phage therapy, and the original home of phage therapy, Georgia, arranges treatment for international patients — reflecting the growing recognition of their use. In 2018, the United States opened the first Western clinical phage base: the Center for Innovative Phage Applications and Therapeutics at the University of California San Diego School of Medicine, and recently received a US Food & Drug Administration approval for a phase I/II clinical trial on intravenous phage use as an adjunct treatment. Clinical use of ‘phage banks’ are also under review, in the hope of speeding up patient care by drawing on a library of pre-approved phage options.
Box 2: Next generation phages
Those not content to harness natural phage potential instead look to enhance them. Two biotech companies are genetically engineering phages with the very weapon that bacteria developed to protect against them: CRISPR-Cas, the bacterial immune system against viruses.
Locus Bioscience, based in North Carolina, United States, equips its phages with a CRISPR-Cas3 add-on, which ‘chews-up’ bacteria DNA. The advantage, it has found, is “a much greater depth of kill. We commonly see increases in killing efficacy of several orders of magnitude”, says Joseph Nixon, vice president of Locus Bioscience.
On the other hand, Eligo Bioscience, based in Paris, France, uses CRISPR-Cas 9, which instead makes precise double-strand DNA cuts that bacteria cannot repair. Going a step further, Eligo removes phage DNA entirely, retaining only the delivery mechanism for its Cas9 gene[10]
. This produces a higher kill rate and circumvents the replication-dependent ‘self-dosing’ issue. By “hijacking” the phage delivery vehicle, “we’re basically bringing gene therapy to the microbiome”, explains Xavier Duportet, co-founder of Eligo Bioscience. “We are delivering a synthetic gene sequence, a payload to the microbiome through the phage capsid,” he explains. This opens options to use phages to carry instructions to switch genes on or off in other bacteria, such as our native gut microbiome, empowering them to target pathogenic bacteria directly — a new-age probiotic.
Future possibilities do not stop at synthetic genes; synthetic phages may also be on the table. Graham Hatfull, professor of biotechnology at the University of Pittsburgh, United States describes the dream of phage therapy; a ‘built-by-design’ phage, where every gene is chosen to enhance its potency and commercial use. “It’s a very attractive idea that, just as the pharmaceutical chemist would design and build a new molecule or a variant of an antibiotic, you would design [a phage] … in an intelligent way, where you have exactly the genes you do want, none of the genes you don’t.”
References
[1] Schooley RT, Biswas B, Gill JJ et al. Antimicrob Agents Chemother 2017;61(10):e00954-17. doi: 10.1128/AAC.00954-17
[2] Chan BK, Sistrom M, Wertz J et al. Scientific Reports 2016;6:26717. doi: 10.1038/srep26717
[3] Chaudhry WN, Concepción-Acevedo J, Park T et al. PLoS One 2017;12(1):e0168615. doi: 10.1371/journal.pone.0168615
[4] Eaton MD & Bayne-Jones S. J Am Med Assoc 1934;103(23):1769–1776. doi: 10.1001/jama.1934.72750490003007
[5] Eaton MD & Bayne-Jones S. J Am Med Assoc 1934;103(24):1847–1853. doi: 10.1001/jama.1934.72750500003009
[6] Eaton MD & Bayne-Jones S. J Am Med Assoc 1934;103(25):1934–1939. doi: 10.1001/jama.1934.72750510005009
[7] Brüssow H. Virology 2012;434(2):138-142. doi: 10.1016/j.virol.2012.09.015
[8] Jault P, Leclerc T, Jennes S et al. Lancet Infect Dis 2019; 9(1):35–45. doi: 10.1016/S1473-3099(18)30482-1
[9] McCallin S, Sacher JC, Zheng J & Chan BK. Viruses 2019;11(4):343. doi: 10.3390/v11040343
[10] Bikard D, Euler C, Jiang W et al. Nature Biotechnology 2014;32:1146–1150. doi: 10.1038/nbt.3043