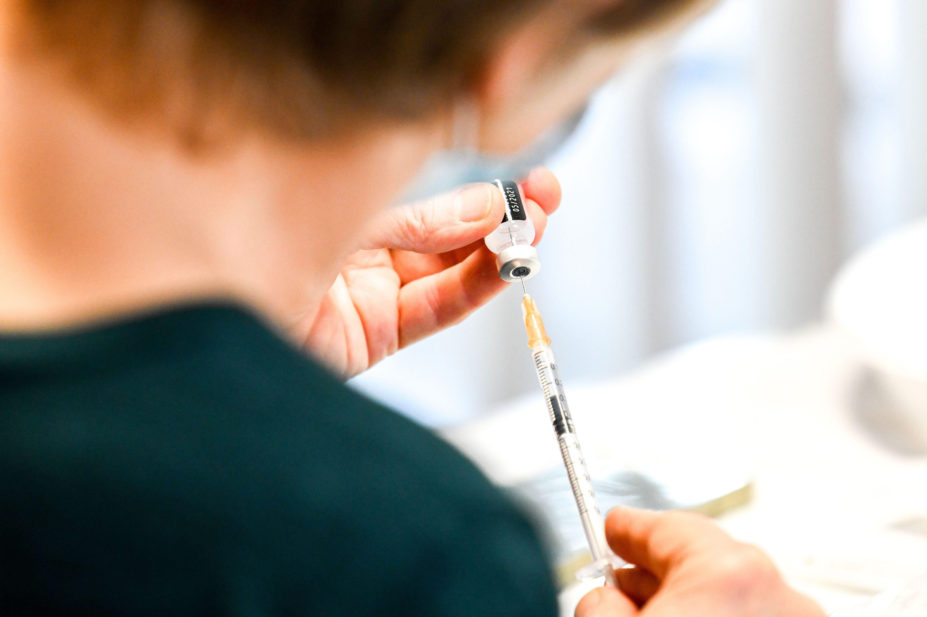
Andia / Alamy Stock Photo
After reading this article, you should be able to:
- Understand the immunological mechanisms of vaccination and the pharmacology of mRNA vaccines;
- Understand the recent advances in mRNA vaccine technology to optimise stability and delivery;
- Identify the clinical uses of mRNA vaccines;
- Advise and educate other healthcare professionals and patients on the background, clinical uses and safety of mRNA vaccines.
Vaccination is one of the most effective public health interventions to prevent, control and even eradicate infectious diseases. It is believed that, with the exception of clean water and sanitation, vaccination has had the greatest contribution of all health interventions to human health and life expectancy[1].
Vaccines can be broadly classified as ‘live’, containing attenuated strains of a microorganism, or ‘non-live’, containing either killed microorganisms or components of a pathogen[2]. Many types of vaccine exist, with the strategy for each presenting its own advantages and disadvantages (see Table 1).
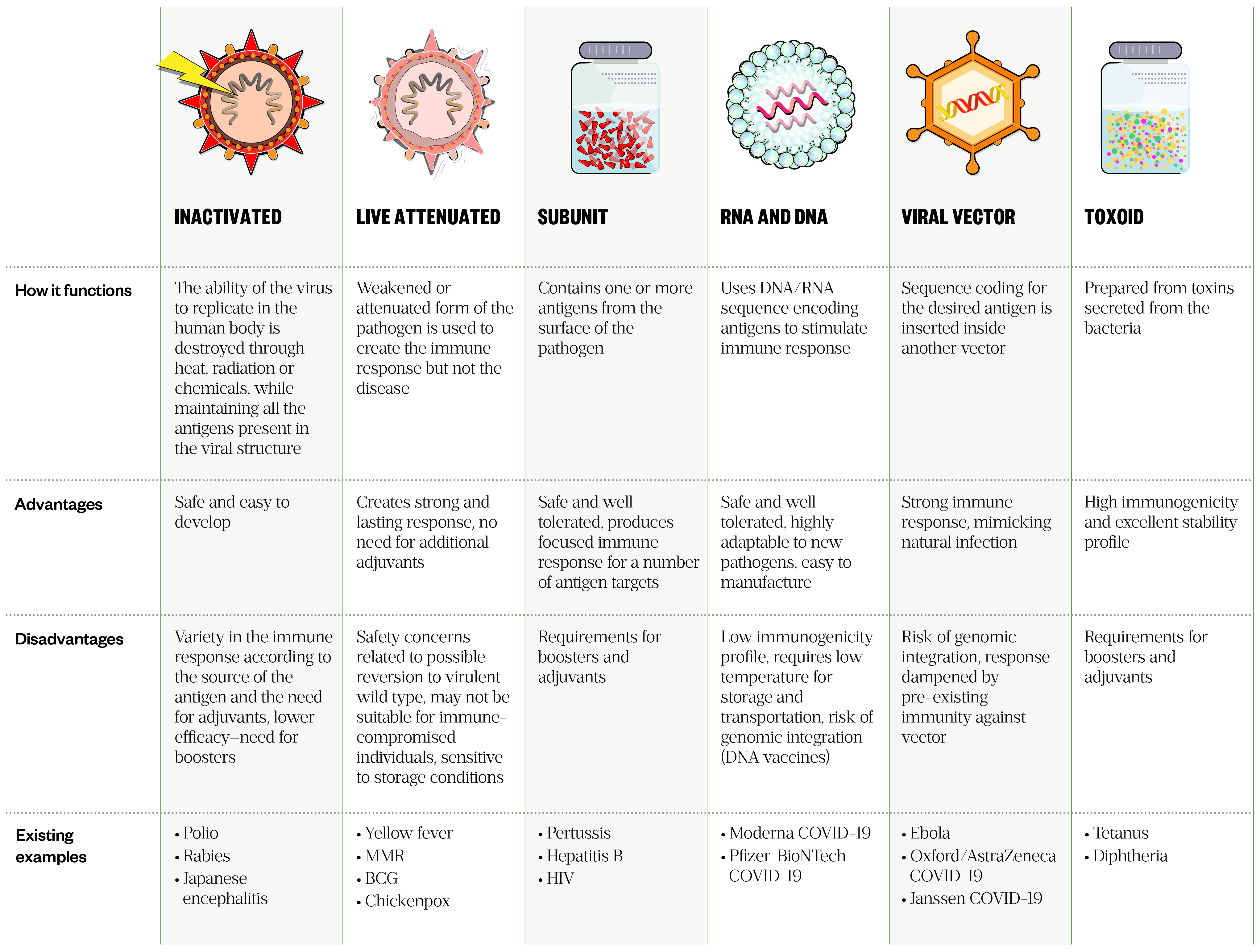
Despite the success of conventional vaccines, significant challenges are still faced at all stages of the research and development process. Prominent challenges have traditionally been the length of time required for, and the financial cost of, each stage of the process[5,6]. When responding to fast-developing infectious disease outbreaks, it is the amount of time required for conventional vaccine development, not the lack of efficacy, which puts conventional vaccines in a disadvantaged position compared with RNA and DNA vaccines[7].
Conventional vaccine constructs (attenuated strains of pathogen or components thereof) are achieved through extensive cell or animal cultures[8]. Therefore, manufacturing attenuated strains inherently involves replication in a foreign host, where the pathogen needs to accumulate mutations to impair its virulence to human hosts[2]. Not only can this process be time-consuming and yield poorly attenuated strains, but when dealing with a virus with high frequency of recombination, such as SARS-CoV-2, it can also lead to recombination of a fully virulent strain[2,9].
Emergent alternatives to traditional vaccines, which use recombinant bacteria or viruses, include genetic vaccines that consist of DNA (as plasmids) or RNA (as messenger RNA, also known as mRNA). These vaccines are taken up by cells and translated into proteins, which then act as intracellular antigens and stimulate an immune response[10,11]. Although the use of mRNA vaccines is only recently — in 2020, following the outbreak of COVID-19 — the first report of successful RNA transfection (introduction of nucleic acid into animal cells) was in 1989 (see Figure 1).
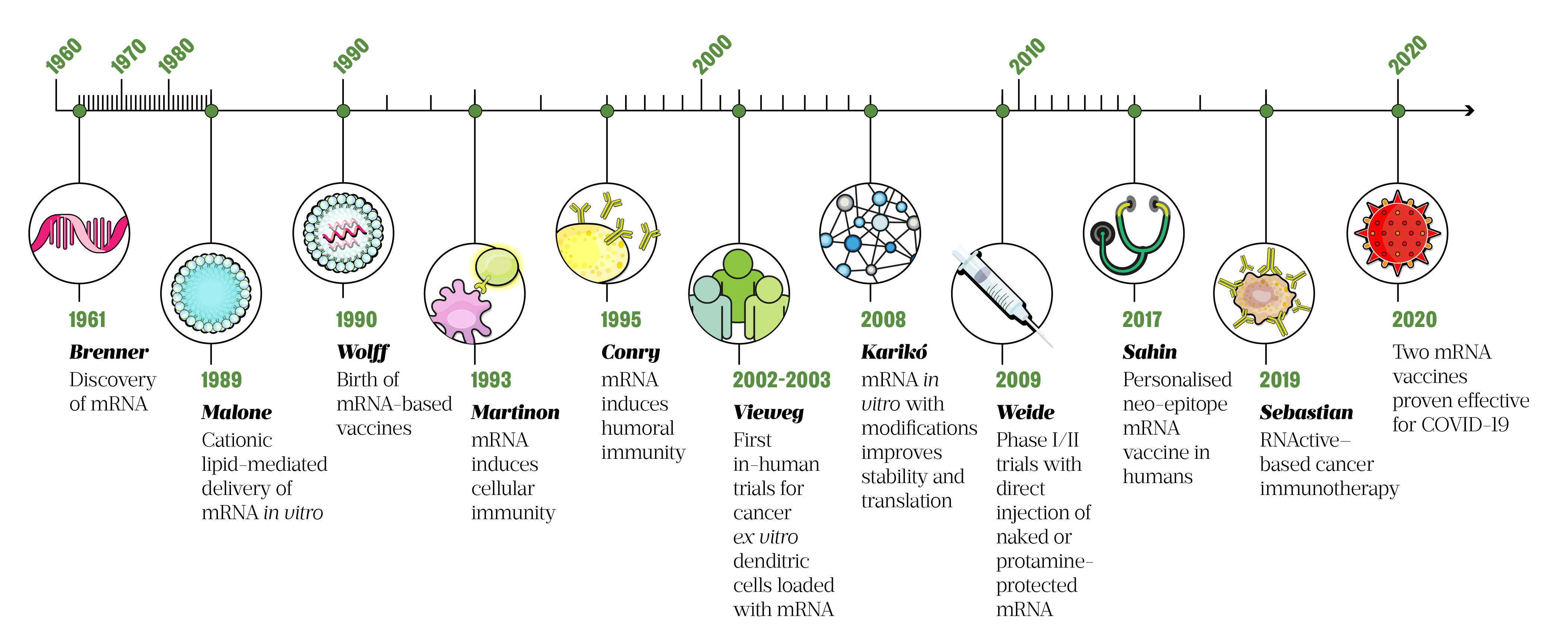
Today, mRNA-based vaccines are a promising technology platform for the development of many therapeutic and prophylactic vaccines. Their manufacturing process is cell-free, safe and time-saving, and avoids the need to grow highly pathogenic organisms on a large scale, reducing the risks of contamination or release of dangerous pathogens. Furthermore, the flexible nature of the mRNA vaccine platform negates the need for a dedicated product-specific production facility. During manufacturing, changes to the encoded protein only alter the sequence of the RNA molecule, meaning the physiochemical characteristics of a mRNA sequence remain largely unaffected. This allows for a more streamlined manufacturing process, accelerating cost-effective mRNA vaccine development and mass production[11]. For many emerging infectious diseases, the main challenge of traditional vaccination techniques is obtaining a stockpile in a short timeframe. Therefore, mRNA vaccines are particularly helpful in a pandemic scenario, where time is critical and vaccines are desperately needed; this has been evidenced in the recent worldwide rollout of the Pfizer-BioNTech and Moderna COVID-19 vaccines, in an unprecedented acceleration of vaccine production[6].
The successful introduction of mRNA vaccines in response to the COVID-19 pandemic has increased interest and focus in mRNA platforms, with many mRNA-based vaccine candidates already in pre-clinical and clinical stages[23]. With the emergence of new mRNA vaccines approved for use, pharmacists have a significant role in educating the public and advocating the uptake of vaccines. Furthermore, pharmacists play a vital role, not only in vaccine distribution but also administration in several countries, such as the UK. This article outlines how mRNA vaccines work, their clinical uses, and how pharmacists can educate and advise other healthcare professionals and patients.
Immunological mechanisms of vaccination
The principle of vaccination is to activate an effective immune response without causing the associated disease. Vaccines do this by inducing active immunity to provide immunological memory. Immunological memory enables the immune system to recognise and respond rapidly to exposure to the same pathogen later on. To achieve this, vaccines contain natural or synthetic antigens, which either consist of, relate to or are derived from the pathogen[24]. A schematic representation of vaccine-induced immunity is shown in Figure 2.
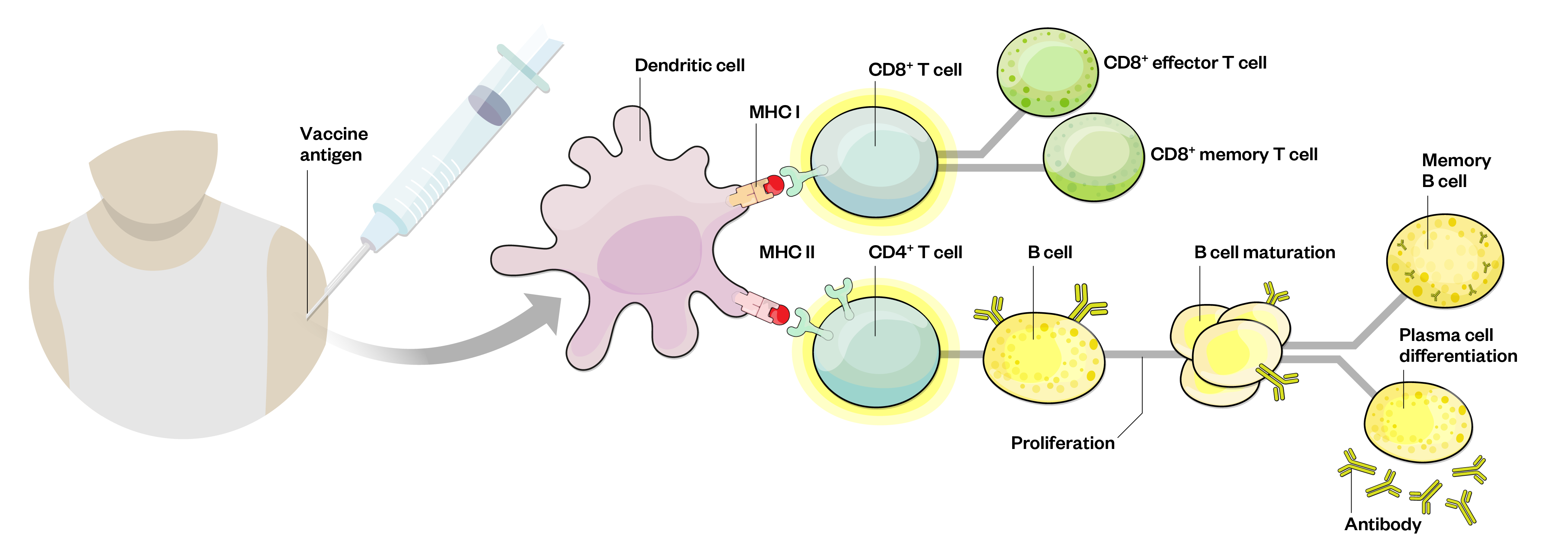
Recognition of antigen at B- and T-cell level leads to proliferation, maturation, differentiation and production of effector T-cells and antibodies[25]
Following immunisation, the vaccine components are taken up by phagocytic cells, such as macrophages and dendritic cells that live in the peripheral tissue. Antigen-presenting cells (APCs) take up antigens and become activated and migrate towards nearby lymph nodes. Inside the lymph nodes, the APCs present the processed antigen to T- and B-lymphocytes, which, under the right conditions (e.g. recognition of the antigen and appropriate co-stimulatory signals), results in the activation of these lymphocytes. These antigen-specific B- and T-cells then clonally expand to produce multiple progenitors that recognise the same antigen. In addition, memory B- and T-cells are formed to provide long-term, sometimes lifelong, protection against infection with the pathogen[24].
Pharmacology of mRNA vaccines
mRNA is the intermediate step between the translation of protein-encoding DNA and the production of proteins by ribosomes in the cytoplasm. The concept behind mRNA vaccines is simple: once the antigen of choice from the pathogen is identified and the gene is sequenced, mRNA is synthesised in vitro from a DNA template[26]. Once inside the cell, the mRNA sequence is translated into the corresponding antigen protein, eliciting cellular and humoral immunity (see Figure 3)[22,27]. Synthetic mRNA is engineered to resemble mature eukaryotic mRNA molecules and generally consists of an open reading frame encoding the target antigen, flanking untranslated regions (UTRs), a five-prime (5’) cap and a terminal poly(A) tail (see Figure 3)[28,29]. The 3’ and 5’ non-coding sequence enhances translational efficacy and boosts mRNA stability[29]. The poly(A) tail also plays a significant regulatory role and prevents degradation of the molecule[30].
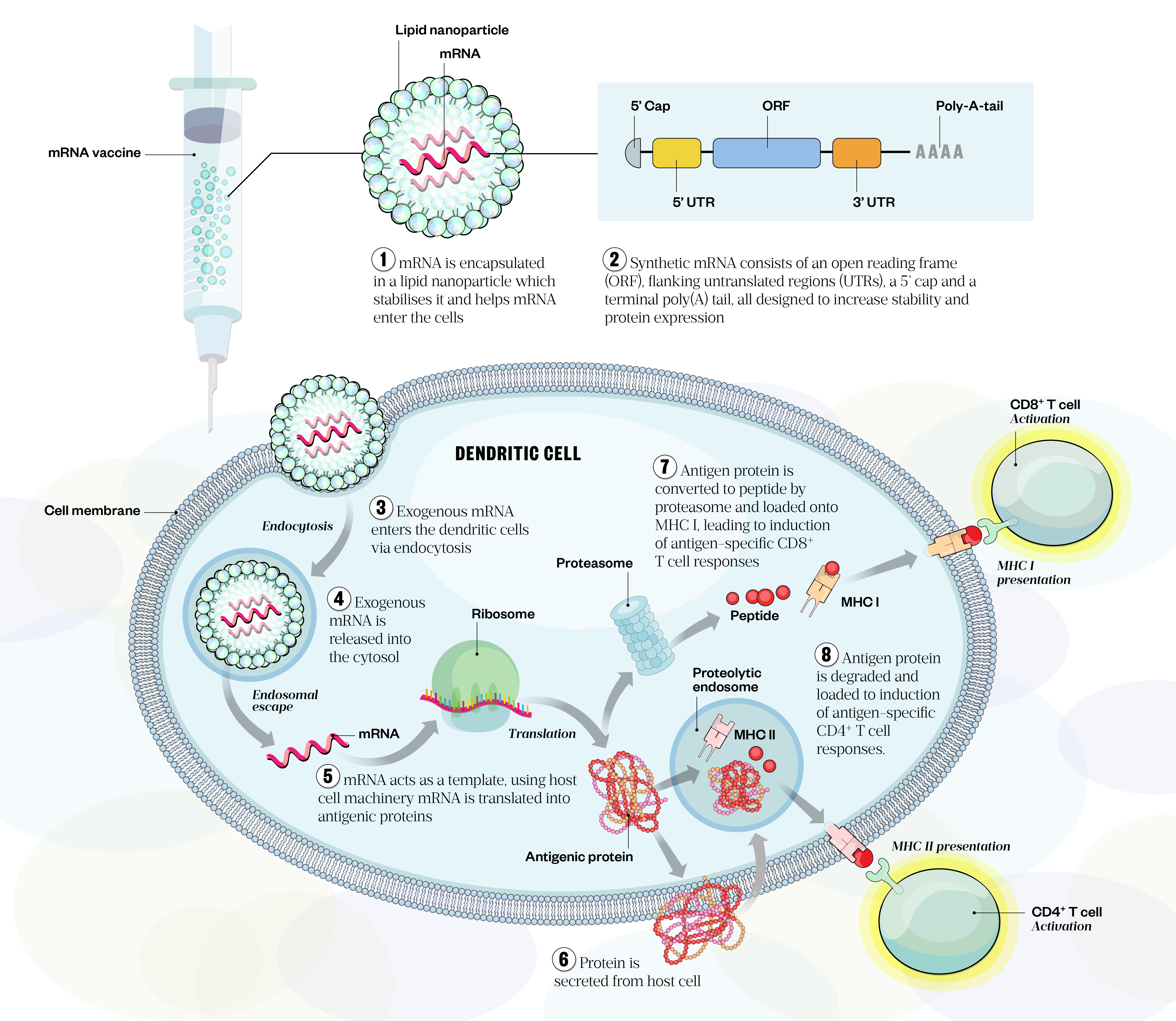
Two general classes of mRNA are currently used in vaccinology: non-replicating mRNA and self-amplifying RNA; current COVID-19 mRNA vaccines belong to the former category. Vaccines based on non-replicating mRNA only encode the antigenic protein of interest, whereas self-amplifying RNA amplifies the immune response by encoding the antigen of interest as well as the viral replication machinery that enables intracellular RNA amplification and abundant protein expression[28,30,31].
Administration routes and delivery strategies for mRNA vaccines
Major administration routes
Several factors influence vaccine efficacy, which can be broadly divided into three categories:
- Vaccine features (i.e. design, formulation, use of adjuvant)
- Individual variation amongst recipients (i.e. age, sex, comorbidity)
- Administration-related parameters (i.e. route of administration and delivery vehicle)[32].
The anatomical and physiological properties of the administration site can affect the safety profile of the vaccine[33]. The choice between systemic or local administration strategies is governed by the antigen expression localisation requirements of mRNA vaccines; for example, intraperitoneal and intravenous routes are employed when systemic expression of the antigen is desirable for therapeutic purposes, whereas intramuscular, subcutaneous and intradermal injections are commonly used routes for mRNA vaccines against infectious diseases (see Table 2)[29].
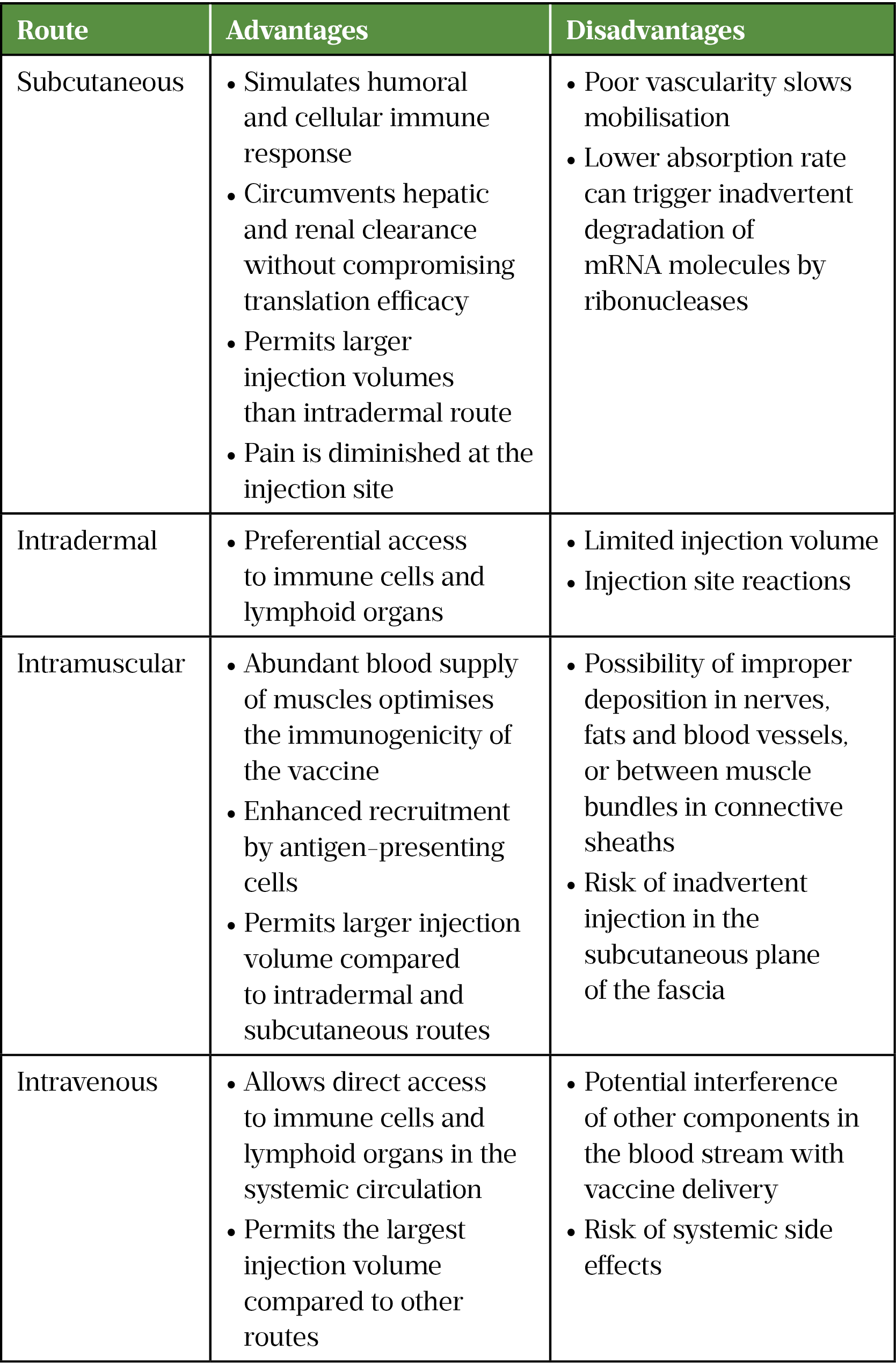
Optimisation of mRNA stability and delivery
One of the major limitations of in vitro transcribed mRNA vaccines is their high innate immunogenicity, where a robust immune response resembling viral invasion is induced, which can produce toll-like receptor-mediated inflammation[41,42]. To negate this problem, modified nucleotides capable of escaping immune system surveillance have been introduced in the mRNA sequence[43]. Indeed, replacement of the nucleosides cytidine, uridine and adenosine with modified analogues, such as 5-methylcytidine, pseudouridine and N6‐methyladenosine, has reduced immunogenicity while enhancing the stability and translation efficiency of the molecule[41].
A major challenge impeding the successful translation of mRNA therapeutics is in vivo delivery. The large size and negative charge of the mRNA molecule hinder membrane permeability. Furthermore, mRNAs are easily degraded by ubiquitous extracellular ribonucleases[44,45]. Encapsulation of mRNA into a carrier is therefore vital to protect the molecule against digestion and confer efficient cellular uptake. Injection of naked mRNA without an additional carrier has been used successfully to target APCs via intranodal injections[46]. However, in the absence of a delivery vehicle, mRNA absorption is severely diminished and the half-life of mRNA is around seven hours.
Ex vivo loading of the dendritic cells with mRNA vaccine allows precise cell-specific delivery and high transfection efficacy, but this labour-intensive costly approach is not a pragmatic technique to vaccination[47]. Delivery systems can generally be divided into viral and non-viral vectors. A summary of advantages and limitations of current delivery systems can be seen in Figure 4. An ideal delivery vehicle protects mRNA from enzymatic digestion, confers cell uptake and triggers endosomal escape and release of the cargo in the cytoplasm[29].
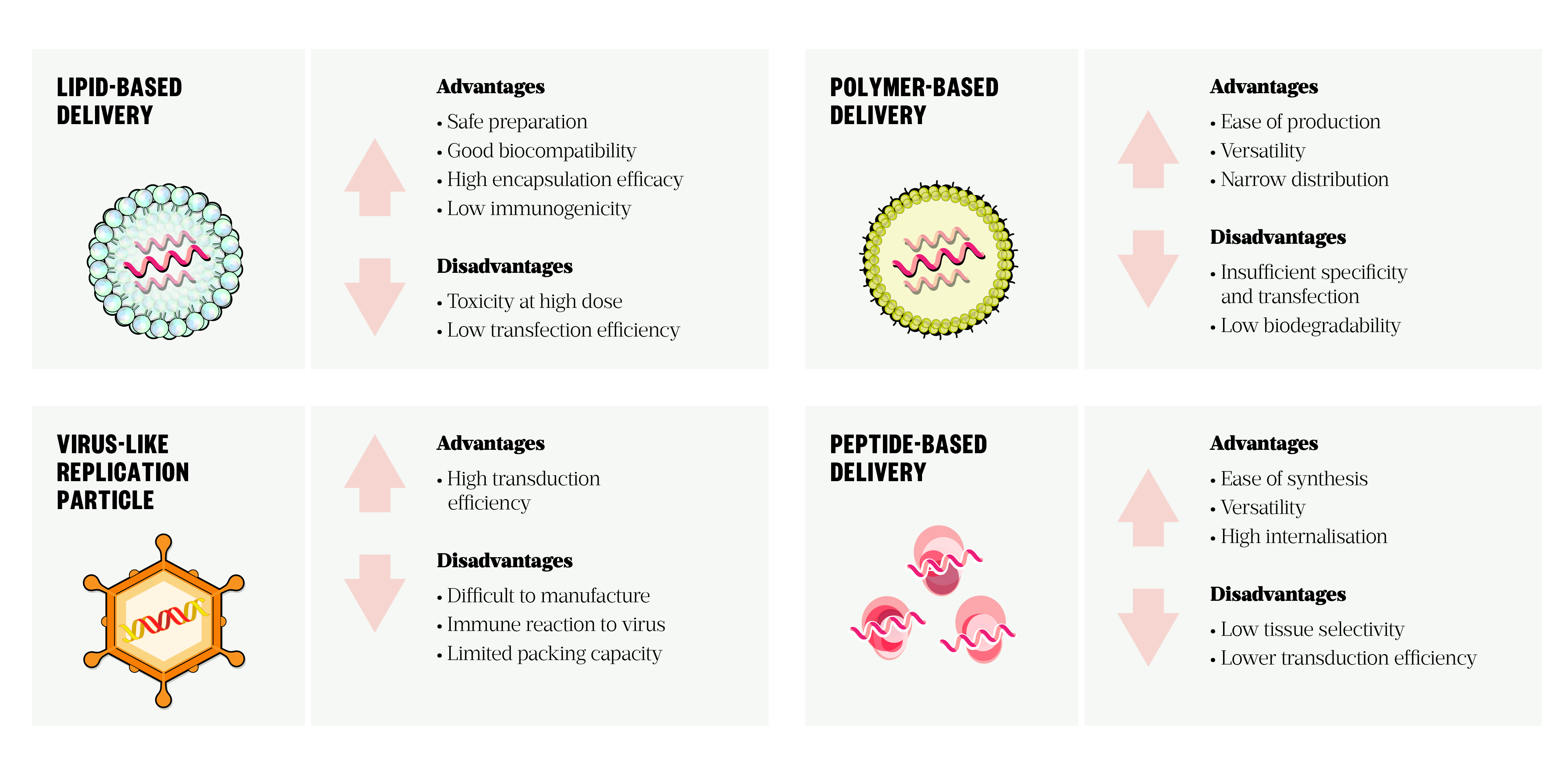
Lipid-based vectors, such as liposomes and lipid nanoparticles (LNPs), are the most widely used mRNA delivery tool[47,53,54]. LNPs consist of four main components:
- Ionisable cationic lipids, which mediate self-assembly, facilitate membrane fusion and promote endosomal escape;
- Lipid-anchored polyethylene glycol (PEG), which increases half-life of the formulation, prolongs circulation time and prevents aggregation by acting as a steric barrier;
- Cholesterol, which acts as a stabilising agent;
- Neutral phospholipids, which support the bilayer structure[55].
Clinical use, safety, limitations and prospects of mRNA vaccines
mRNA vaccines have a promising safety profile. Advances in mRNA design, nucleic acid delivery technologies and the cell-free manufacturing process have minimised the risks associated with nucleic acid-based vaccines[56]. However, rare cases of severe local and systemic reactions have been reported[57,58]. Moreover, further studies are needed to investigate biodistribution and persistence of immunogen[47]. Induction of potent type I interferon responses associated with inflammation and autoimmunity have been reported in a few nucleic acid-based vaccine studies[59–61].
Another potential safety issue stems from the presence of extracellular RNA leading to an increase in the permeability of endothelial cells, which can contribute to oedema. Furthermore, extracellular RNA can serve as promoters of blood coagulation and pathological thrombus formation[62]. Therefore, longitudinal studies are required to monitor and assess the safety profile of these vaccines, and understand the durability of immunity[63].
A higher incidence of anaphylactic reactions has been reported with the Pfizer-BioNTech COVID-19 vaccine (around 4.7 per 1 million vaccinations) and the Moderna COVID-19 vaccine (around 2.5 per 1 million vaccinations) which is about two- to four-fold more than traditional vaccines[63,64]. It has been hypothesised that PEGylated lipid used in the nanoparticles of these vaccines is the reason, owing to the presence of pre-existing antibodies against PEG in some individuals as a result of exposure to PEG present in many consumer products, such as toothpastes, shampoos and laxatives[65]. Table 3 lists commonly reported side effects for COVID-19 mRNA vaccines.
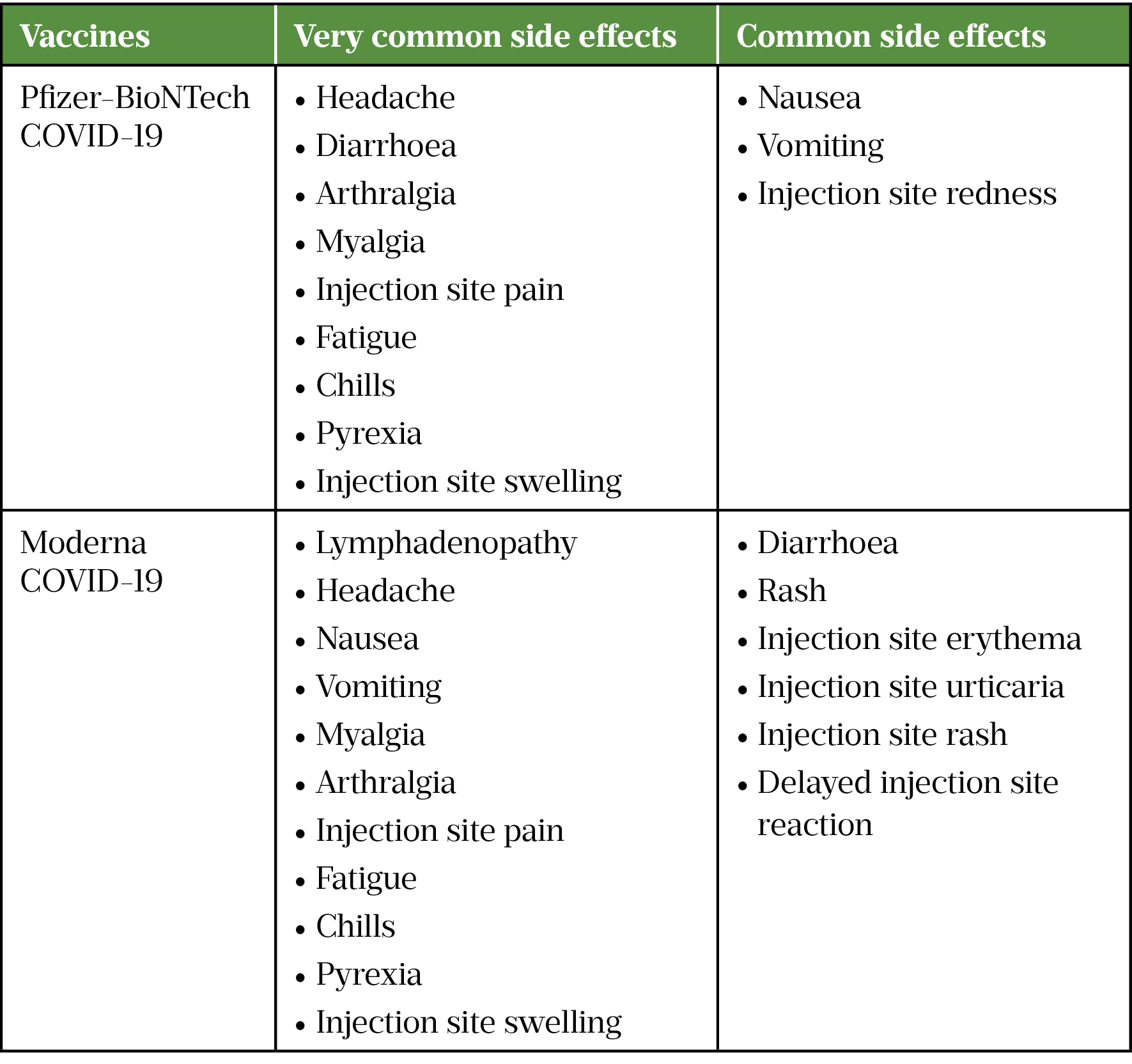
Challenges around mRNA vaccines are interlinked and exacerbated in a pandemic situation. Some of these challenges are:
- Cold-chain storage, which affects distribution of the vaccines worldwide, particularly in warm countries or those without reliable cold-chain storage. This also impacts stability and degradation of the mRNA vaccine. One strategy to address this may be changing the formulation, paying special consideration to mRNA size, guanine-cytosine and lipid content;
- Emerging viral variants, where some mutations in the viral genome can enhance immune evasion and reduce cross-variant efficacy of mRNA vaccines, resulting in the need for variant-specific mRNA boosters;
- Duration of antibody response, where a decline in antibody titres have been witnessed over time. The durability of the response will vary from one antigen to another and warrants longer-term data collection;
- Vaccine acceptance, where public mistrust fuelled by misinformation can jeopardise maintenance of herd immunity when a rapid response is needed to eradicate the viral infection of interest. Pharmacists have an important role in improving vaccine acceptance by addressing myths around vaccination, especially around mRNA vaccines (see Figure 5 for myths around mRNA vaccines and facts that can be used as counselling points)[56].
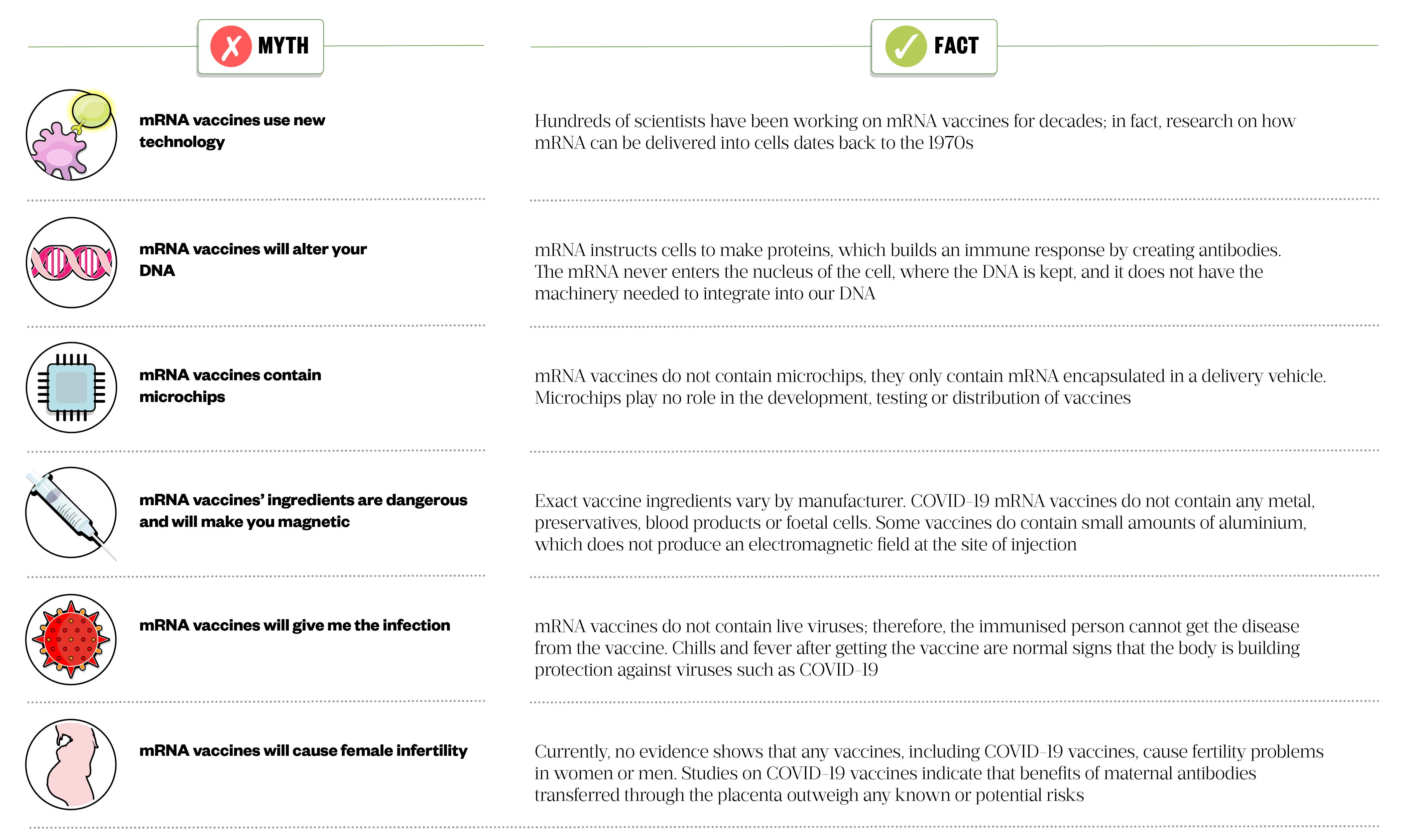
Many publications have explored the application of mRNA vaccines against viral and tumoral targets[41]. According to data analytics company GlobalData, there are currently 23 out of 44 ongoing mRNA vaccine trials related to infectious diseases[69]. Although clinical evaluation is still limited, development of mRNA vaccines against a wide range of infectious diseases, such as zika virus, influenza, rabies, Ebola, HIV and respiratory syncytial virus is currently being explored[56,70].
The SARS-CoV-2 pandemic gave prominence to mRNA-based vaccines. The fast manufacturing pace, minimalist nature, high immunogenicity and ease of modification resulted in an unprecedented speed in vaccine development, where clinical testing of the first mRNA vaccine candidate began only 66 days after SARS-CoV-2 sequencing data was made public[71].
mRNA-based cancer vaccines hold great promise for the treatment of malignancies where tumour-associated and tumour-specific neoepitopes unique to cancerous cells can be targeted. The majority of cancer vaccines are therapeutic rather than prophylactic and seek to shrink and destroy tumours by simulating cell-mediated responses[72]. Furthermore, they are non-oncogenic, well-tolerated and capable of delivering large amounts of patient-specific antigens, making them an attractive form of cancer immunotherapy[73].
A limited number of studies have applied mRNA vaccine technology towards combating bacterial and parasitic diseases. However, the structural and immunological complexities that stem from multiplex bacterial composition and parasites’ reproduction cycles complicate the choice of vaccine antigen. Furthermore, the majority of bacterial and parasitic infections are treatable using low-cost antibiotics and antiparasitic drugs, diminishing the need for vaccine development[41].
Summary
With the recent worldwide approval of mRNA vaccines against COVID-19, the future for mRNA vaccines is undoubtedly bright. Nevertheless, further insights into their mechanism of action, potency and long-term safety are needed. Continued advancement in mRNA formulation and delivery should accelerate mRNA vaccine development.
- 1Greenwood B. The contribution of vaccination to global health: past, present and future. Phil. Trans. R. Soc. B. 2014;369:20130433. doi:10.1098/rstb.2013.0433
- 2Kyriakidis NC, López-Cortés A, González EV, et al. SARS-CoV-2 vaccines strategies: a comprehensive review of phase 3 candidates. npj Vaccines. 2021;6. doi:10.1038/s41541-021-00292-w
- 3Li Y-D, Chi W-Y, Su J-H, et al. Coronavirus vaccine development: from SARS and MERS to COVID-19. J Biomed Sci. 2020;27. doi:10.1186/s12929-020-00695-2
- 4Chatterjee R, Ghosh M, Sahoo S, et al. Next-Generation Bioinformatics Approaches and Resources for Coronavirus Vaccine Discovery and Development—A Perspective Review. Vaccines. 2021;9:812. doi:10.3390/vaccines9080812
- 5Kaufmann SH, Juliana McElrath M, Lewis DJ, et al. Challenges and responses in human vaccine development. Current Opinion in Immunology. 2014;28:18–26. doi:10.1016/j.coi.2014.01.009
- 6Rosa SS, Prazeres DMF, Azevedo AM, et al. mRNA vaccines manufacturing: Challenges and bottlenecks. Vaccine. 2021;39:2190–200. doi:10.1016/j.vaccine.2021.03.038
- 7Verbeke R, Lentacker I, De Smedt SC, et al. Three decades of messenger RNA vaccine development. Nano Today. 2019;28:100766. doi:10.1016/j.nantod.2019.100766
- 8Heaton PM. Challenges of Developing Novel Vaccines With Particular Global Health Importance. Front. Immunol. 2020;11. doi:10.3389/fimmu.2020.517290
- 9Tao Y, Shi M, Chommanard C, et al. Surveillance of Bat Coronaviruses in Kenya Identifies Relatives of Human Coronaviruses NL63 and 229E and Their Recombination History. J Virol. 2017;91. doi:10.1128/jvi.01953-16
- 10Leitner WW, Ying H, Restifo NP. DNA and RNA-based vaccines: principles, progress and prospects. Vaccine. 1999;18:765–77. doi:10.1016/s0264-410x(99)00271-6
- 11Armbruster, Jasny, Petsch. Advances in RNA Vaccines for Preventive Indications: A Case Study of A Vaccine Against Rabies. Vaccines. 2019;7:132. doi:10.3390/vaccines7040132
- 12BRENNER S, JACOB F, MESELSON M. An Unstable Intermediate Carrying Information from Genes to Ribosomes for Protein Synthesis. Nature. 1961;190:576–81. doi:10.1038/190576a0
- 13Malone RW, Felgner PL, Verma IM. Cationic liposome-mediated RNA transfection. Proceedings of the National Academy of Sciences. 1989;86:6077–81. doi:10.1073/pnas.86.16.6077
- 14Wolff JA, Malone RW, Williams P, et al. Direct Gene Transfer into Mouse Muscle in Vivo. Science. 1990;247:1465–8. doi:10.1126/science.1690918
- 15Martinon F, Krishnan S, Lenzen G, et al. Induction of virus-specific cytotoxic T lymphocytesin vivo by liposome-entrapped mRNA. Eur. J. Immunol. 1993;23:1719–22. doi:10.1002/eji.1830230749
- 16Conry R, LoBuglio A, Wright M, et al. Characterization of a messenger RNA polynucleotide vaccine vector. Cancer Res 1995;55:1397–400.https://www.ncbi.nlm.nih.gov/pubmed/7882341
- 17Heiser A, Coleman D, Dannull J, et al. Autologous dendritic cells transfected with prostate-specific antigen RNA stimulate CTL responses against metastatic prostate tumors. J. Clin. Invest. 2002;109:409–17. doi:10.1172/jci0214364
- 18Karikó K, Buckstein M, Ni H, et al. Suppression of RNA Recognition by Toll-like Receptors: The Impact of Nucleoside Modification and the Evolutionary Origin of RNA. Immunity. 2005;23:165–75. doi:10.1016/j.immuni.2005.06.008
- 19Weide B, Pascolo S, Scheel B, et al. Direct Injection of Protamine-protected mRNA: Results of a Phase 1/2 Vaccination Trial in Metastatic Melanoma Patients. Journal of Immunotherapy. 2009;32:498–507. doi:10.1097/cji.0b013e3181a00068
- 20Sahin U, Derhovanessian E, Miller M, et al. Personalized RNA mutanome vaccines mobilize poly-specific therapeutic immunity against cancer. Nature. 2017;547:222–6. doi:10.1038/nature23003
- 21Sebastian M, Schröder A, Scheel B, et al. A phase I/IIa study of the mRNA-based cancer immunotherapy CV9201 in patients with stage IIIB/IV non-small cell lung cancer. Cancer Immunol Immunother. 2019;68:799–812. doi:10.1007/s00262-019-02315-x
- 22Xu S, Yang K, Li R, et al. mRNA Vaccine Era—Mechanisms, Drug Platform and Clinical Prospection. IJMS. 2020;21:6582. doi:10.3390/ijms21186582
- 23Chakraborty C, Sharma AR, Bhattacharya M, et al. From COVID-19 to Cancer mRNA Vaccines: Moving From Bench to Clinic in the Vaccine Landscape. Front. Immunol. 2021;12. doi:10.3389/fimmu.2021.679344
- 24Jiskoot W, Kersten GFA, Mastrobattista E, et al. Vaccines. Pharmaceutical Biotechnology. 2019;:281–304. doi:10.1007/978-3-030-00710-2_14
- 25Pollard AJ, Bijker EM. A guide to vaccinology: from basic principles to new developments. Nat Rev Immunol. 2020;21:83–100. doi:10.1038/s41577-020-00479-7
- 26Maruggi G, Zhang C, Li J, et al. mRNA as a Transformative Technology for Vaccine Development to Control Infectious Diseases. Molecular Therapy. 2019;27:757–72. doi:10.1016/j.ymthe.2019.01.020
- 27Okay S, Özge Özcan Ö, et al. Nanoparticle-based delivery platforms for mRNA vaccine development. AIMS Biophysics. 2020;7:323–38. doi:10.3934/biophy.2020023
- 28Schlake T, Thess A, Fotin-Mleczek M, et al. Developing mRNA-vaccine technologies. RNA Biology. 2012;9:1319–30. doi:10.4161/rna.22269
- 29Zhang C, Maruggi G, Shan H, et al. Advances in mRNA Vaccines for Infectious Diseases. Front. Immunol. 2019;10. doi:10.3389/fimmu.2019.00594
- 30Wadhwa A, Aljabbari A, Lokras A, et al. Opportunities and Challenges in the Delivery of mRNA-Based Vaccines. Pharmaceutics. 2020;12:102. doi:10.3390/pharmaceutics12020102
- 31Vogel AB, Lambert L, Kinnear E, et al. Self-Amplifying RNA Vaccines Give Equivalent Protection against Influenza to mRNA Vaccines but at Much Lower Doses. Molecular Therapy. 2018;26:446–55. doi:10.1016/j.ymthe.2017.11.017
- 32Zhang L, Wang W, Wang S. Effect of vaccine administration modality on immunogenicity and efficacy. Expert Review of Vaccines. 2015;14:1509–23. doi:10.1586/14760584.2015.1081067
- 33Zeng C, Zhang C, Walker PG, et al. Formulation and Delivery Technologies for mRNA Vaccines. Current Topics in Microbiology and Immunology. 2020. doi:10.1007/82_2020_217
- 34De Beuckelaer A, Grooten J, De Koker S. Type I Interferons Modulate CD8 + T Cell Immunity to mRNA Vaccines. Trends in Molecular Medicine. 2017;23:216–26. doi:10.1016/j.molmed.2017.01.006
- 35Golombek S, Pilz M, Steinle H, et al. Intradermal Delivery of Synthetic mRNA Using Hollow Microneedles for Efficient and Rapid Production of Exogenous Proteins in Skin. Molecular Therapy – Nucleic Acids. 2018;11:382–92. doi:10.1016/j.omtn.2018.03.005
- 36Krebs J, Goldstein E, Kilpatrick S. Lewin’s Genes XII. Burlington, Massachusetts, United States: : Jones & Bartlett Learning 2017.
- 37Gradel AKJ, Porsgaard T, Lykkesfeldt J, et al. Factors Affecting the Absorption of Subcutaneously Administered Insulin: Effect on Variability. Journal of Diabetes Research. 2018;2018:1–17. doi:10.1155/2018/1205121
- 38Zuckerman JN. The importance of injecting vaccines into muscle. BMJ. 2000;321:1237–8. doi:10.1136/bmj.321.7271.1237
- 39Diehl K-H, Hull R, Morton D, et al. A good practice guide to the administration of substances and removal of blood, including routes and volumes. J. Appl. Toxicol. 2001;21:15–23. doi:10.1002/jat.727
- 40Reichmuth AM, Oberli MA, Jaklenec A, et al. mRNA vaccine delivery using lipid nanoparticles. Therapeutic Delivery. 2016;7:319–34. doi:10.4155/tde-2016-0006
- 41Wang Y, Zhang Z, Luo J, et al. mRNA vaccine: a potential therapeutic strategy. Mol Cancer. 2021;20. doi:10.1186/s12943-021-01311-z
- 42Roncati L, Corsi L. Nucleoside‐modified messenger RNA COVID‐19 vaccine platform. Journal of Medical Virology. 2021;93:4054–7. doi:10.1002/jmv.26924
- 43Weissman D, Karikó K. mRNA: Fulfilling the Promise of Gene Therapy. Molecular Therapy. 2015;23:1416–7. doi:10.1038/mt.2015.138
- 44Gupta SK, Haigh BJ, Griffin FJ, et al. The mammalian secreted RNases: Mechanisms of action in host defence. Innate Immun. 2012;19:86–97. doi:10.1177/1753425912446955
- 45Sahin U, Karikó K, Türeci Ö. mRNA-based therapeutics — developing a new class of drugs. Nat Rev Drug Discov. 2014;13:759–80. doi:10.1038/nrd4278
- 46Kreiter S, Selmi A, Diken M, et al. Intranodal Vaccination with Naked Antigen-Encoding RNA Elicits Potent Prophylactic and Therapeutic Antitumoral Immunity. Cancer Res. 2010;70:9031–40. doi:10.1158/0008-5472.can-10-0699
- 47Pardi N, Hogan MJ, Porter FW, et al. mRNA vaccines — a new era in vaccinology. Nat Rev Drug Discov. 2018;17:261–79. doi:10.1038/nrd.2017.243
- 48Thomas TJ, Tajmir-Riahi H-A, Pillai CKS. Biodegradable Polymers for Gene Delivery. Molecules. 2019;24:3744. doi:10.3390/molecules24203744
- 49Park S-Y, Kim K-H, Kim S, et al. BMP-2 Gene Delivery-Based Bone Regeneration in Dentistry. Pharmaceutics. 2019;11:393. doi:10.3390/pharmaceutics11080393
- 50Dizaj SM, Jafari S, Khosroushahi AY. A sight on the current nanoparticle-based gene delivery vectors. Nanoscale Res Lett. 2014;9. doi:10.1186/1556-276x-9-252
- 51Reissmann S. Cell penetration: scope and limitations by the application of cell-penetrating peptides. J. Pept. Sci. 2014;20:760–84. doi:10.1002/psc.2672
- 52Liang Y, Huang L, Liu T. Development and Delivery Systems of mRNA Vaccines. Front. Bioeng. Biotechnol. 2021;9. doi:10.3389/fbioe.2021.718753
- 53Corthésy B, Bioley G. Lipid-Based Particles: Versatile Delivery Systems for Mucosal Vaccination against Infection. Front. Immunol. 2018;9. doi:10.3389/fimmu.2018.00431
- 54Li B, Zhang X, Dong Y. Nanoscale platforms for messenger RNA delivery. WIREs Nanomed Nanobiotechnol. 2018;11. doi:10.1002/wnan.1530
- 55Cao Y, Gao GF. mRNA vaccines: A matter of delivery. EClinicalMedicine. 2021;32:100746. doi:10.1016/j.eclinm.2021.100746
- 56Chaudhary N, Weissman D, Whitehead KA. mRNA vaccines for infectious diseases: principles, delivery and clinical translation. Nat Rev Drug Discov. 2021;20:817–38. doi:10.1038/s41573-021-00283-5
- 57Alberer M, Gnad-Vogt U, Hong HS, et al. Safety and immunogenicity of a mRNA rabies vaccine in healthy adults: an open-label, non-randomised, prospective, first-in-human phase 1 clinical trial. The Lancet. 2017;390:1511–20. doi:10.1016/s0140-6736(17)31665-3
- 58Pardi N, Tuyishime S, Muramatsu H, et al. Expression kinetics of nucleoside-modified mRNA delivered in lipid nanoparticles to mice by various routes. Journal of Controlled Release. 2015;217:345–51. doi:10.1016/j.jconrel.2015.08.007
- 59Nestle FO, Conrad C, Tun-Kyi A, et al. Plasmacytoid predendritic cells initiate psoriasis through interferon-α production. Journal of Experimental Medicine. 2005;202:135–43. doi:10.1084/jem.20050500
- 60Theofilopoulos AN, Baccala R, Beutler B, et al. TYPE I INTERFERONS (α/β) IN IMMUNITY AND AUTOIMMUNITY. Annu. Rev. Immunol. 2005;23:307–35. doi:10.1146/annurev.immunol.23.021704.115843
- 61Edwards DK, Jasny E, Yoon H, et al. Adjuvant effects of a sequence-engineered mRNA vaccine: translational profiling demonstrates similar human and murine innate response. J Transl Med. 2017;15. doi:10.1186/s12967-016-1111-6
- 62Kannemeier C, Shibamiya A, Nakazawa F, et al. Extracellular RNA constitutes a natural procoagulant cofactor in blood coagulation. Proceedings of the National Academy of Sciences. 2007;104:6388–93. doi:10.1073/pnas.0608647104
- 63Rahman MA, Islam MS. Early approval of COVID-19 vaccines: Pros and cons. Human Vaccines & Immunotherapeutics. 2021;17:3288–96. doi:10.1080/21645515.2021.1944742
- 64McNeil MM, Weintraub ES, Duffy J, et al. Risk of anaphylaxis after vaccination in children and adults. Journal of Allergy and Clinical Immunology. 2016;137:868–78. doi:10.1016/j.jaci.2015.07.048
- 65Vrieze J. Suspicions grow that nanoparticles in Pfizer’s COVID-19 vaccine trigger rare allergic reactions. Science. 2020. doi:10.1126/science.abg2359
- 66Summary of Product Characteristics for Spikevax. Medicines & Healthcare products Regulatory Agency. 2021.https://www.gov.uk/government/publications/regulatory-approval-of-covid-19-vaccine-moderna/information-for-healthcare-professionals-on-covid-19-vaccine-moderna (accessed Mar 2022).
- 67Summary of Product Characteristics for COVID-19 Vaccine Pfizer/BioNTech. Medicines & Healthcare products Regulatory Agency. 2021.https://www.gov.uk/government/publications/regulatory-approval-of-pfizer-biontech-vaccine-for-covid-19/summary-of-product-characteristics-for-covid-19-vaccine-pfizerbiontech (accessed Mar 2022).
- 68COVID-19 mRNA Vaccine BNT162b2 concentrate for solution for injection. Electronic medicines compendium. 2021.https://www.medicines.org.uk/emc/product/12634/smpc#gref (accessed Mar 2022).
- 69mRNA vaccines: the post-pandemic outlook after a breakthrough year. Pharmaceutical Technology. 2021.https://www.pharmaceutical-technology.com/features/mrna-vaccines-pandemic-outlook/ (accessed Mar 2022).
- 70Nitika, Wei J, Hui A-M. The Development of mRNA Vaccines for Infectious Diseases: Recent Updates. IDR. 2021;Volume 14:5271–85. doi:10.2147/idr.s341694
- 71Bettini E, Locci M. SARS-CoV-2 mRNA Vaccines: Immunological Mechanism and Beyond. Vaccines. 2021;9:147. doi:10.3390/vaccines9020147
- 72Mehmet C. Messenger RNA Vaccines: Beckoning of a New Era in Cancer Immunotherapy. ONCOLOGY. 2021. doi:10.46883/onc.2021.3504.0198
- 73McNamara MA, Nair SK, Holl EK. RNA-Based Vaccines in Cancer Immunotherapy. Journal of Immunology Research. 2015;2015:1–9. doi:10.1155/2015/794528